Neutrinos
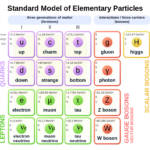
Neutrinos are fermions (spin 1/2) in the standard model, so named because they are electrically neutral, and have very little mass (hence the -ino, meaning small). Since they are electrically neutral, they cannot interact via the electromagnetic force, and since they don’t have color, they don’t interact via the strong nuclear force either. They are known to only interact via the weak force and gravity.
Neutrino Interactions
Since neutrinos have non-zero mass, they will interact ever-so-slightly via gravity. That being said, because their mass is so low, gravity is not usually part of talks on neutrino interactions. They are however an important player in nuclear weak force interactions. The weak force is most closely related to the electromagnetic force in the sense that it has to do with electric charge, however there are a couple main differences. One difference between the two forces is the force-propagating bosons of the weak force have mass and therefore only happen over shorter distances, and instead of simply acting between two charged particles, the weak force can not only act between charged particles, but act by transferring the charge from one body to another via charged bosons. Lastly, the weak force does not have to preserve the generation of the lepton. For example, if an electron neutrino interacts with a proton, the outgoing neutrino does not have to remain an electron neutrino. It could be a muon neutrino instead.
Force-Propagating Bosons
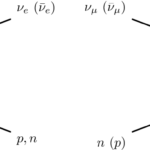
Charge Current: The W+/- bosons are the charged vector bosons and are responsible for transferring charge away from one initial particle to a final state particle. In neutrino-nucleon interactions, these bosons are responsible for transferring charge from the nucleon (proton/neutron) to a final state lepton not necessarily matching the flavor of the initial neutrino. See the right side of the figure above for an example. Notice how some negative charge from the neutron is transferred away, allowing it to become a proton, while the charge is transferred into an electron final state. Note that the incoming neutrino was a muon neutrino, while the outgoing lepton was an electron.
Neutrino Oscillations
Like the other leptons, neutrinos come in three generations: electron, muon and tau. Unlike the other leptons, neutrinos have the unique ability to oscillate between generations, muon neutrinos can spontaneously change to tau or electron for example. While the timing of a neutrino oscillation is completely random and unpredictable, the state of a neutrino beam can be predicted/calculated as the rates of these changes are known empirically.
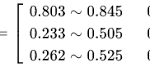
The matrix above is known as the PMNS matrix and gives the mixing rates for each generation neutrino into every other. If you know the distance your neutrino beam will be travelling, and what it looks like at the source, you can calculate the makeup of the beam at any other point.
LArTPC Mechanics
There are a couple components of note in an LArTPC detector.
Liquid Argon
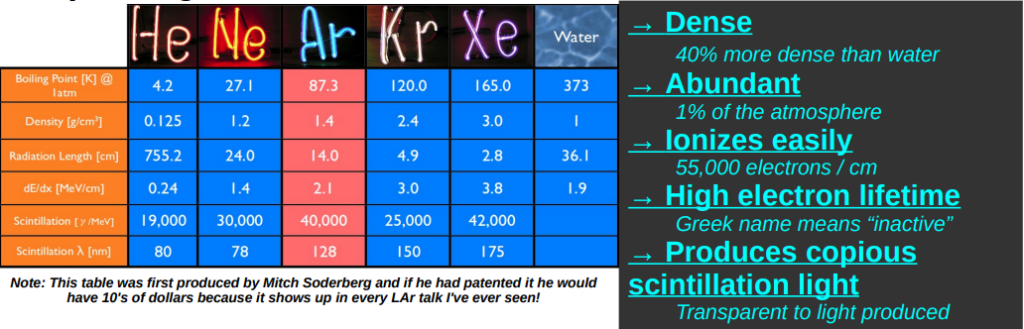
Detectors of this type have been made with many different materials. All of which are shown in the table above. Liquid argon is an excellent choice for large TPC detectors relative to the other available options.
Time Projection Chamber
The nature of a TPC detector is a tank of material that ionizes as a particle travels through it. When subjected to an electric field, the electrons that were knocked free from ionization will travel at a constant velocity towards the detector wall. By recording a location and the time that the electrons took to reach the detector wall, you can calculate a x,y,z position of the charge when it first knocked free. By doing this over the entire side of the detector, TPCs are able to reconstruct particle events in three dimensions. DUNE is currently being built with wire readout electronics where two overlapping planes of perpendicular wires (on the order of 1.5×10^6 wires), each reading out with 100MHz precision. This produces a lot of data (exobytes each year) and most of it is trash (just zeros), so its a waste of space.
DUNE Overview
Deep Underground Neutrino Experiment (DUNE) is an international flagship neutrino experiment involving 2 state-of-the-art detectors in a neutrino beam produced by FermiLab. DUNE ND (for Near Detector) is a high resolution, modular Liquid Argon Time Projection Chamber (LArTPC) detector right at the beam source used to characterize the neutrino beam. DUNE FD (for Far Detector) is a 40kton fiducial mass LArTPC detector a mile underground in South Dakota’s Sanford Underground Research Facility. DUNE FD is approximately 1300km away from the beam source at an optimal position to study electron neutrinos.
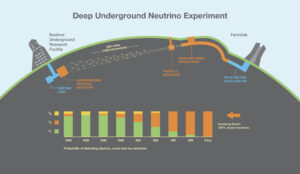
DUNE – Putting It All Together
When a neutrino initially enters DUNE FD, it will be invisible as it is not charged, and therefore does not cause scintillation, but also does not interact with anything so it will not cause ionization either. We only are aware of the neutrino if it happens to interact with an electron or nucleus in the fiducial region. Once it does, it will cause an interaction producing many particles, some of which are charged and therefore cause scintillation, and all of which interact more with the medium around them, and will cause ionization along their path. Each type of particle has a dE/dx (an amount of charge radiated in the form of ionization) specific to the particle, its energy and the medium. As seen in the plot above (and with more sig figs), LAr produces 42000 electrons per MeV deposited ionization energy. For an illustration, a muon has a dE/dx around 2MeV/cm in LAr. Over the course of 10 cm, the muon has deposited 20MeV of energy into the LAr medium. At a rate of 42000e-/MeV, this suggests that over the 10cm, the muon has freed 840000 e-. These now free charges will travel (drift) in the electric field in the -z direction towards the detector wall. At the same time, the electron swarm will diffuse both longitudinally (in the direction of motion) and transversely (orthogonal to the direction of motion). We know the drift velocity of electrons in LAr under a 500V/cm electric field is basically constant, with a value of 1.618mm/us. We also know that diffusion of the swarm can be calculated as \sigma_{L/T} = \sqrt{\frac{2zD_{L/T}}{v_{e-}^3}}, where \sigma_{L/T} is the rms of the Gaussian shaped diffused swarm in either longitudinal or transverse directions respectively, D_{L/T} is a diffusion constant, z is the drift distance (or distance between where the particle track occurred and the detector wall), and finally v_{e-} is the drift velocity of the electrons in LAr. Based on where the particle was, and the drift velocity, we can calculate how long it will take for the electrons to reach the detector wall, and how spread out the swarm is when it gets there.
There are some complications however. First, we are not actually given where the particle is. In simulations, we call this kind of information “truth”. It is the real answer that we start with, and simulate responses based off of, and then try to reproduce, but technically in real life, we are not able to access it. The closest we get is being able to accurately and precisely reproduce the truth with the simulated data and trust we are doing the same thing with the real data.
Determining a t0
In order to reproduce the truth, we need to find a way to locate the event in the fiducial region. Since we know that electrons drift at a more or less constant velocity, we can calculate the z by using the drift velocity and the difference in t (when we detect the electron at the detector wall) and t0 (when the electron was originally knocked free by the particle). Since we don’t have an inherent t0 measurement like we have a t measurement, we need to get creative in figuring it out.
However we don’t know when the event (the initial creation of the electron swarm) actually occurred. By doing all the analysis we’ve been talking about, we find the cleanest pixel with a reasonable number of resets and use it to calculate a t0. Then, when we reconstruct, we have 2 options:
1) use the rms of the charge swarm in each pixel to calculate a tof and then a z
2) use the constant drift velocity
Since the rms of the charge swarm depends on a clean gaussian distribution, any deviation from that (or multiple deposits) will throw off the calculation, and resolution worsens as a function of Z – were talking a centimeter or so at low z’s and 10s of cm at higher z’s. Its very tough to be sure of measurements, and preserve the shape of the event (which I imagine is important)
Since the drift velocity is constant, regardless of the Z, we can use it to calculate a z to within a cm or so. Once we have a t0, using a precise method like the drift velocity to calculate the z will preserve the shape of the event. Most of the uncertainty is going to be in the offset, since it is dictated by a measurement from the rms.
Light Detection Method
The light detection method is more self-explanatory than the lightless method. This method depends on light’s ability to travel faster than anything else (and much much faster than electrons can drift through LAr). Essentially when a charged particle travels through LAr, it causes the LAr to scintillate (produce photons). LAr produces a lot of photons (see the chart above) which is one of the reasons it makes such a good TPC medium. Since light from the scintillation will reach the detector wall almost immediately (approx 10 nanoseconds compared to 100s of microseconds) we can incorporate a light detection method, and as long as we can calibrate it to match the scintillation light from an event to its corresponding electron swarms, we have a t0 and a t, and can use it to calculate z. Since the scintillation light also follows the event, there is more you can learn from the light beyond a t0. More work is being dedicated to this concept currently in the Q-Pix group.
Lightless (Charge-Only) Method
Another interesting way to calculate the t0 of an event comes from the concept that the electron swarm diffuses at a calculable rate. By rearranging the equation for longitudinal diffusion, we can use the constant drift velocity, the longitudinal diffusion constant, and the rms of the Gaussian shaped electron swarm in time to figure out a z (or a t because t = z/v_{e-}). By doing this, we remove the need for light detection, or at least add another way of checking the validity and accuracy of the light based measurements.
Tracking and Vertexing
The next step to reconstructing events is to actually put the pieces back together. Based on where the electrons are detected on the wall, we get a x and a y, and the z that is calculated in the above steps gives us the final piece. From there, its a matter of connecting the dots. We have a collection of points in x,y, and z, but they need to be connected in the right way to accurately reflect the interactions in the event. In other words, we need to recreate the particle tracks and interaction vertexes in order to correctly determine the type of event and flavors/parameters of initial particles.
Charge Pixels (Q-Pix)
DUNE is currently designed to have readout technology involving millions of wires per module, each reading out with GPS precision. This ends up resulting in exobytes of data each year. The kicker is on top of this huge data output rate, since neutrinos barely interact, most of it is going to be empty – just a bunch of zeros where nothing was happening, but the detector is still reading out. This requires huge data storage capacity and also countless man hours to sort through the garbage and find important data. Saving the day from this terrible data problem is the Q-Pixel (more commonly referred to as Q-Pix, standing for charge pixel). This new readout technology is designed to output a signal if there is something happening, and if not, do nothing. What this does is instead of getting years worth of data filled with zeros that you need to sort through, this will only give you information when there is information to be had.
The Q-Pix is designed to be a charge detecting pixel, connected to something called an Charge Integrate-Reset (CIR) block. This piece of circuitry is designed to build charge up to a certain threshold, at which point it will output a pulse, connect the bridge and let the charge buildup equilibrate before starting again. This is what the group calls a “reset”. Now instead of having to follow each individual electron, we only see a reset when a certain threshold has been reached. That being said, we can do many things with these, like record the time between resets. Knowing a reset occurs after a certain amount of charge has been collected, and knowing the time between resets allows us to calculate the current, and ultimately go back and more or less reconstruct the original shape of the electron cloud that the pixel detected in the first place.
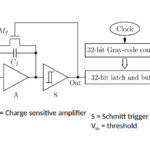
Resets are in essence just a fancy word for down sampling. Since each reset stands for a set amount of electrons – in this case 6250 – we can treat them like really big electrons and treat them the same way as the original wire readout technology treated individual electrons. The rms and mean measurements of the thousands of electrons that are in the electron swarms can still be done with the tens of resets in each pixel. Since a pixel is inherently 2 dimensional, by reading the data out of a single pixel, we already know the x and y without needing to compare it to other wires or pixels. and since the pixel is 4mm x 4mm, we know that x and y down to a 4mm precision. This will help with events with complex topologies.
The goal of the Q-Pix collaboration is to show that Q-Pix is a worst matching the performance of the originally designed wire readout electronics, while drastically reducing the data output from EB to TB.
What we can study with DUNE/QPix
Supernova Neutrinos
Supernovae are some of the most powerful events in the universe. They are some of the brightest as well. Even though these giant explosions can be big and bright enough to be seen with the naked eye, we are still blind to what goes on inside the collapsing star as it happens. Because of their extremely weak coupling to matter and their near-c speeds, neutrinos are actually able to reach the earth before the light from a supernova (the light gets trapped in the extremely dense collapsing star). Because of this, neutrino detectors are able to study the profile of neutrinos emitted from the star as it collapses to get a more detailed view of the inner mechanics than we could ever get studying them view telescope. Also, being able to wire the many neutrino detectors around the world to search for neutrino events that look like the start of a supernova allows us to have an essentially planet sized supernova detector that gives us a heads up that a supernova is occurring before we even get to see it.
The Q-Pix group is investing time and effort into exploring the capabilities of Q-Pix when dealing with supernova neutrinos.
Beam Neutrinos
DUNE is being built in line with one of the brightest neutrino sources in the world. It is safe to say, this experiment was made to look at high energy neutrino events.
Nucleon Decay
Some models suggest that protons are actually not entirely stable, and have the ability to decay just like other unstable particles. The only difference between protons and other more unstable particles is that the lifetime of the proton is expected to be on the order of 10^34 years (in other words, that is 10^24 times the current age of the universe). Its safe to say this interaction hasn’t happened often, if at all. That being said, if it does occur, it is EXTREMELY important that we catch it. The best way to catch something that almost never happens is to make the biggest target we can and give it the best opportunity. This is why DUNE, an experiment the size of a four story building filled with LAr is one of the best chances we have to see this. If we do see something like this, we will be able to say a lot about the Standard Model and our current understandings of the universe.
Phenomenology
Just because Q-Pix is a technology we are developing and testing for use with DUNE FD, doesn’t mean Q-Pix cannot have other applications. For example, DUNE is an experiment made with a unique sensitivity to electron neutrinos and antineutrinos. This is because the distance of the detector from the source corresponds to a point where neutrino oscillation has produced a peak amount of electron favored neutrinos. While this makes it very good for studying some interactions, others like the weak mixing angle are actually impossible to do with that setup.
The Q-Pix group is also focusing on applications of Q-Pix in different experimental setups with different parameters. The goal is to see if Q-Pix is good enough to have big impacts in other either existing or potentially future experiments.